NASA’s James Webb Space Telescope is the largest and most powerful telescope ever launched to space. Its mirror is composed of 18 individual segments that have been aligned so accurately, that they effectively work as a single giant (21.6-foot, or 6.5-meter) reflector. The process of adjusting each of these separately functioning hexagonal mirror segments requires constant oversight from a dedicated team of engineers and optics scientists. We invite Dr. Marcio B. Meléndez, principal astronomical optics scientist for Webb at the Space Telescope Science Institute, to tell us more about the challenges of aligning the telescope after launch, and what is required to keep it that way during scientific operations.
“Soon after the successful launch and deployment of Webb, an intricate process of aligning its large golden mirrors began. It took nearly three months to go from the initial deployments of 18 individual unfocused segments that had just flown to space, to a completely aligned system bounded only by the optical design.
“Though the precise alignment of the telescope was completed in early 2022 during commissioning, it does not stay that way naturally due to various factors such as temperature variations and so-called ‘tilt’ events, so a lifelong maintenance program is required. The wavefront sensing team responsible for keeping Webb’s mirrors in order has been monitoring, investigating, trending, and occasionally moving its primary mirror segments during science operations. These activities are carried out from Webb’s Mission Operations Center, located at the Space Telescope Science Institute in Baltimore.
“This telescope monitoring program consists of a series of observations that use special optical sensing equipment inside the Near Infrared Camera instrument (NIRCam), with a set of lenses that intentionally defocus the images of stars by a known amount. These defocused star images contain measurable features that enable the team to derive the alignment of the telescope, using a process called phase retrieval to determine what we call the ‘wavefront error.’ The telescope monitoring observations are currently scheduled every other day, interspersed among Webb’s science observations, with short runtimes of about 20 minutes. All telescope monitoring observations are publicly available via the MAST archive. Observatory users and other interested investigators can also view and model the optical quality using specialized tools.
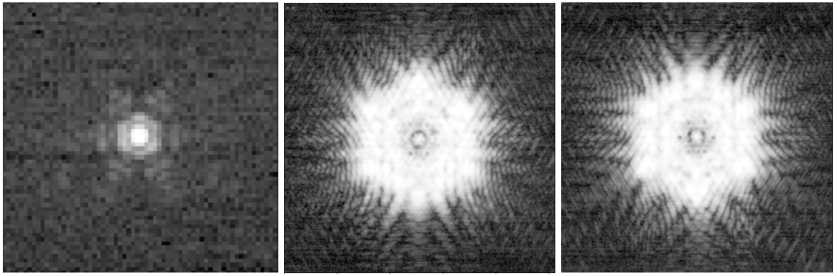
“The maintenance program also takes a ‘selfie’ using a specialized ‘pupil imaging’ lens, designed to take images of the mirror segments and not of the sky, four times a year. These pupil images are used to assess the health of the primary mirrors. During each observation the team measures Webb’s pointing stability or ‘jitter,’ which has remained six times better than design requirements. The Fine Guidance Sensor is used to command a small onboard steerable mirror to lock onto a target, while moving in orbit, without deviating more than the thickness of a human hair, seen at a distance of seven miles (11 kilometers).
“The overall optical performance of the telescope is far better than the design requirement, meaning the observations are even more sensitive to faint objects, and more discerning of fine features than was expected. The optical requirement for Webb was set to 150 nanometers of wavefront error, coming from a combination of uncorrectable surface figure imperfections and correctable telescope misalignments. The current uncorrectable errors are very low, at about 65 nanometers The telescope alignment program aims to achieve and maintain this, and when the observed misalignments accumulate above predetermined criteria, the primary mirror segments are commanded and the system is realigned.
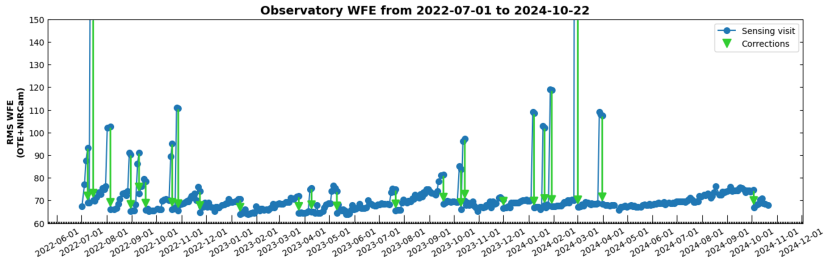
“Each segment from the primary mirror can be repositioned in six ‘degrees of freedom,’ meaning six different types of movement. A segment’s curved surface can also be changed somewhat to adjust its focal length. The Webb telescope mirrors maintain passive alignment through stable support from the backplane structure. As Webb points to different locations in the sky, the heat absorbed from the Sun changes, causing small (0.1 kelvins) temperature changes on the support structure that drive small physical movements. These tiny displacements cause mirror misalignments. This distortion is very small and accounts for only a few nanometers of change in the wavefront. In addition to this, there are sudden offsets to the structure that we call tilt events. These distinct jumps do not reverse themselves, and our current understanding of these events is that they are associated with small but abrupt releases of energy that was stored in the mirror support structure.
“The telescope mirror control updates were required to be less frequent than every two weeks. When a telescope misalignment is observed, the telescope team makes a correction within 48 hours following a well-coordinated procedure between different flight systems. During this time, we create a set of mirror movements intended to re-align the segments. These movements are transformed into commands that are then uploaded and executed. After applying these corrective moves, a new set of observations is taken to confirm the alignment of the telescope. Since the beginning of science operations, we have applied over 25 corrective moves. A time series of all wavefront measurements and the corresponding segment offsets is shown in Figure 3. On Oct. 3, a mirror correction was performed, after a record of 186 days since the previous mirror control update.
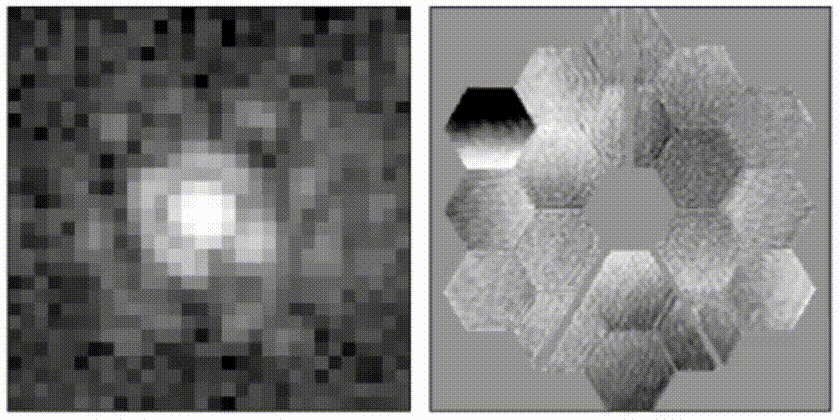
“With the rigorous overall maintenance program of measurement and control, the wavefront team ensures Webb’s optical performance is at the highest possible level to uncover the hidden mysteries of the universe.”
About the author: Dr. Marcio B. Meléndez is a principal astronomical optics scientist at the Space Telescope Science Institute. He is a member of the wavefront sensing team in the telescope branch at STScI.
The fact that Webb’s mirror alignment has required fewer corrections than anticipated not only provides more observation time to conduct Webb science, but also offers important takeaways for future missions. Fewer adjustments indicates better than expected telescope stability, which will be a crucial consideration for missions like NASA’s future Habitable Worlds Observatory. The Habitable Worlds Observatory will be the first space telescope designed to search for life as we know it on Earth-sized planets around nearby Sun-like stars, while exploring many broader transformative astrophysics questions that will reveal secrets of the universe.